BadassBlues
Well-Known Member
This looks promising!
www.universitycube.net
Key Takeaways
3/26/2025
Aa
Medicine
A groundbreaking discovery from UCLA scientists is poised to transform the landscape of hair restoration. Researchers have unveiled a molecule, PP405, which has shown remarkable potential in clinical trials to awaken dormant yet undamaged hair follicles, promising a new frontier in the treatment of pattern baldness. This revelation comes at a time when current FDA-approved treatments, such as minoxidil and finasteride, remain constrained by limited efficacy, benefiting only a fraction of patients. The introduction of PP405 offers hope for a more robust and inclusive approach to combating hair loss, a condition that affects millions globally and carries profound emotional and social ramifications.
Hair loss, particularly androgenetic alopecia or pattern baldness, has long been a vexing challenge for both patients and medical professionals. Despite advances in dermatology, existing treatments often fall short, leaving many to explore supplemental therapies such as red light therapy, platelet-rich plasma injections, or costly hair transplantation procedures. These options, while innovative, are neither curative nor universally accessible, often requiring significant time and financial investment. Against this backdrop, UCLA’s discovery of PP405 represents a potentially transformative leap forward.
The molecule’s mechanism is rooted in the unique metabolic activity of hair follicle stem cells, which play a critical role in hair growth cycles. Scientists have long understood that these stem cells can become dormant due to aging, hormonal changes, or other environmental factors, leading to thinning hair or complete baldness. PP405, however, appears capable of reactivating these inactive cells, effectively jumpstarting the process of hair regrowth. Early clinical trials have demonstrated promising results, with patients experiencing noticeable improvements in hair density and coverage.
What sets PP405 apart is its ability to complement existing therapies rather than replace them. For individuals already using minoxidil or finasteride, the molecule could amplify the efficacy of these treatments, offering a synergistic effect that enhances overall outcomes. This adaptability positions PP405 as a versatile tool in the expanding arsenal of hair restoration solutions, which increasingly emphasize personalized approaches tailored to individual needs.
The timing of this breakthrough is particularly noteworthy, as demand for hair restoration services continues to surge. Experts liken the growing accessibility of modern therapies to the rise of Botox in cosmetic medicine—once a niche procedure, now a mainstream preventive care option. This parallel underscores a cultural shift in attitudes toward aesthetic interventions, where proactive measures are increasingly embraced to preserve youthfulness and confidence.
However, the promise of PP405 also highlights the importance of early intervention and evidence-based treatment strategies. Dermatologists and researchers alike stress the need for patients to seek professional consultation at the first signs of hair thinning, rather than relying on over-the-counter solutions or unproven remedies. Timely diagnosis and treatment not only improve outcomes but also mitigate the psychological impact of hair loss, which can erode self-esteem and social comfort.
Beyond its immediate clinical implications, the development of PP405 signals a broader evolution in the science of regenerative medicine. Hair restoration, once dismissed as a superficial concern, is now recognized as a legitimate field of medical inquiry, intersecting with stem cell research, metabolic studies, and pharmaceutical innovation. The molecule’s success could pave the way for similar advancements in other areas of regenerative therapy, from skin rejuvenation to organ repair.
Moreover, the societal implications of accessible hair restoration therapies warrant consideration. As treatments like PP405 become more widely available, they could democratize the pursuit of aesthetic enhancements, leveling the playing field for individuals across socioeconomic boundaries. At the same time, the normalization of such interventions raises questions about beauty standards and the pressures to conform to ideals of youth and vitality.
In the end, UCLA’s discovery of PP405 is more than a scientific milestone—it is a testament to the power of curiosity and innovation to address deeply human concerns. While the molecule’s journey from laboratory to widespread application is still unfolding, its potential to reshape the narrative of hair loss is undeniable. For those who have long sought solutions to the challenges of pattern baldness, PP405 offers not just hope, but the possibility of reclaiming something profoundly personal—the confidence to feel comfortable in one’s own skin.
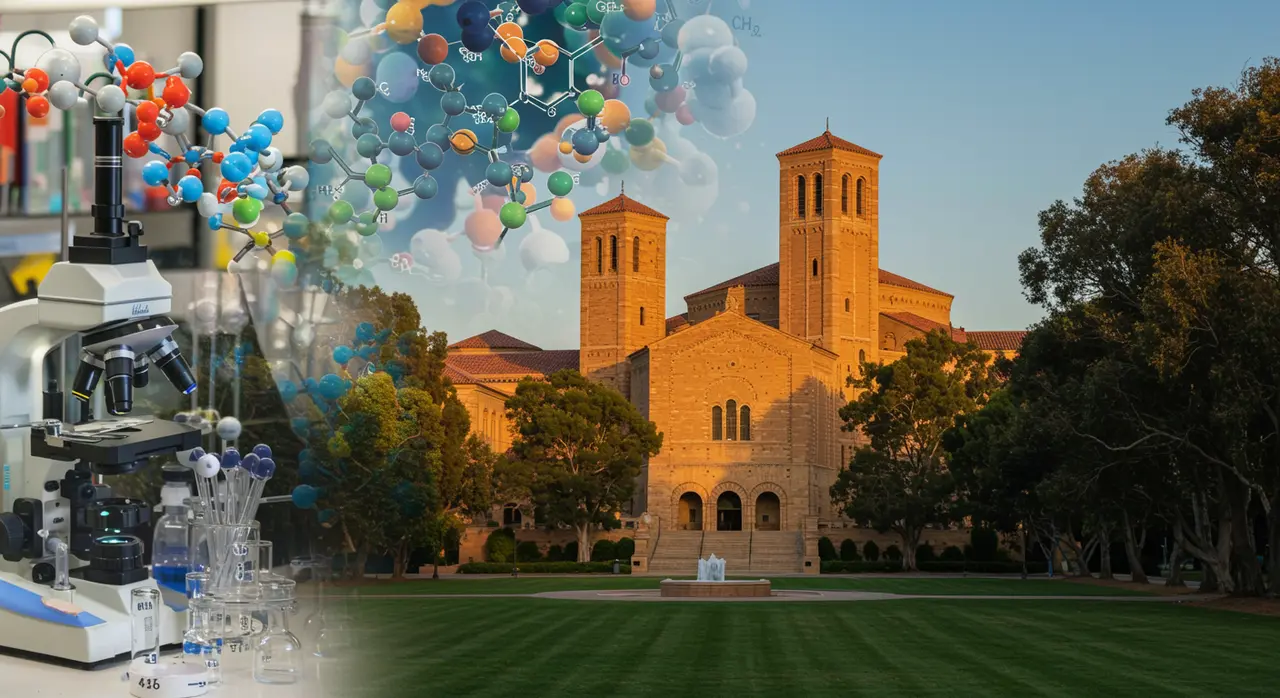
UCLA Scientists Unveil PP405 Molecule, Promising Breakthrough in Hair Restoration Therapy | University Cube
UCLA scientists have unveiled PP405, a molecule that reactivates dormant hair follicle stem cells, offering a groundbreaking and potentially transformative solution for millions affected by pattern baldness globally.
UCLA Scientists Unveil PP405 Molecule, Promising Breakthrough in Hair Restoration Therapy
200 viewsKey Takeaways
3/26/2025
Aa
Medicine
A groundbreaking discovery from UCLA scientists is poised to transform the landscape of hair restoration. Researchers have unveiled a molecule, PP405, which has shown remarkable potential in clinical trials to awaken dormant yet undamaged hair follicles, promising a new frontier in the treatment of pattern baldness. This revelation comes at a time when current FDA-approved treatments, such as minoxidil and finasteride, remain constrained by limited efficacy, benefiting only a fraction of patients. The introduction of PP405 offers hope for a more robust and inclusive approach to combating hair loss, a condition that affects millions globally and carries profound emotional and social ramifications.
Hair loss, particularly androgenetic alopecia or pattern baldness, has long been a vexing challenge for both patients and medical professionals. Despite advances in dermatology, existing treatments often fall short, leaving many to explore supplemental therapies such as red light therapy, platelet-rich plasma injections, or costly hair transplantation procedures. These options, while innovative, are neither curative nor universally accessible, often requiring significant time and financial investment. Against this backdrop, UCLA’s discovery of PP405 represents a potentially transformative leap forward.
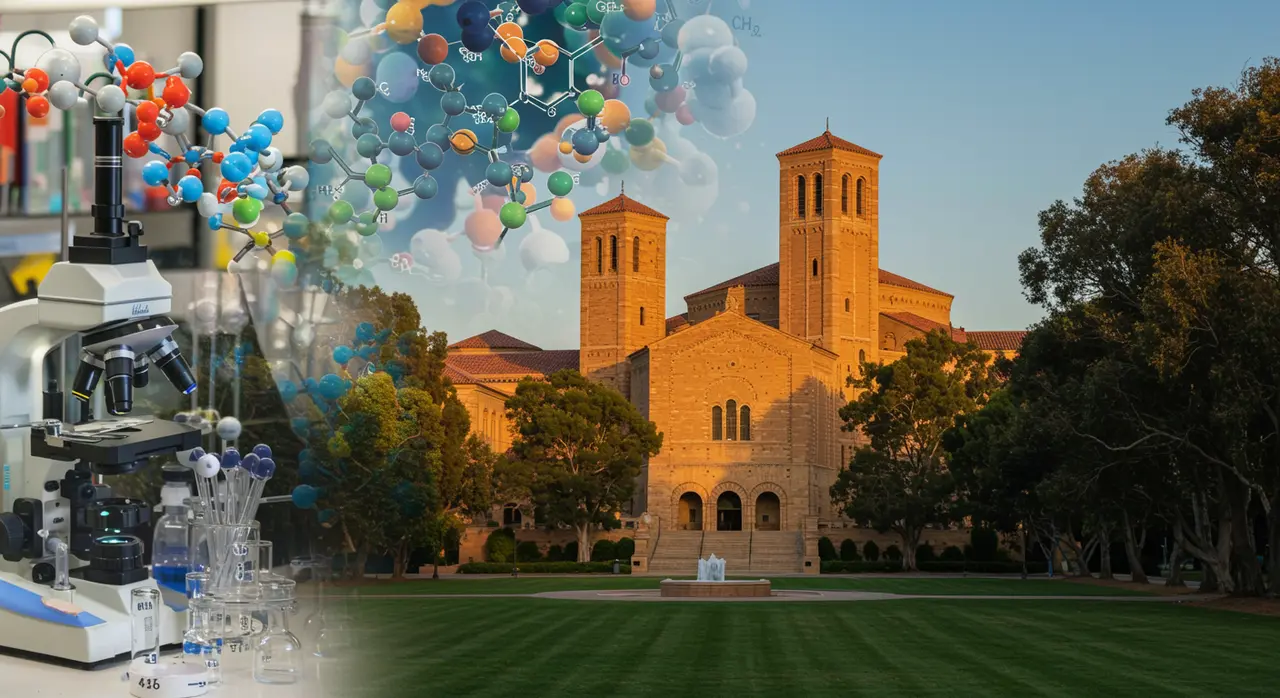
The molecule’s mechanism is rooted in the unique metabolic activity of hair follicle stem cells, which play a critical role in hair growth cycles. Scientists have long understood that these stem cells can become dormant due to aging, hormonal changes, or other environmental factors, leading to thinning hair or complete baldness. PP405, however, appears capable of reactivating these inactive cells, effectively jumpstarting the process of hair regrowth. Early clinical trials have demonstrated promising results, with patients experiencing noticeable improvements in hair density and coverage.
What sets PP405 apart is its ability to complement existing therapies rather than replace them. For individuals already using minoxidil or finasteride, the molecule could amplify the efficacy of these treatments, offering a synergistic effect that enhances overall outcomes. This adaptability positions PP405 as a versatile tool in the expanding arsenal of hair restoration solutions, which increasingly emphasize personalized approaches tailored to individual needs.
The timing of this breakthrough is particularly noteworthy, as demand for hair restoration services continues to surge. Experts liken the growing accessibility of modern therapies to the rise of Botox in cosmetic medicine—once a niche procedure, now a mainstream preventive care option. This parallel underscores a cultural shift in attitudes toward aesthetic interventions, where proactive measures are increasingly embraced to preserve youthfulness and confidence.
However, the promise of PP405 also highlights the importance of early intervention and evidence-based treatment strategies. Dermatologists and researchers alike stress the need for patients to seek professional consultation at the first signs of hair thinning, rather than relying on over-the-counter solutions or unproven remedies. Timely diagnosis and treatment not only improve outcomes but also mitigate the psychological impact of hair loss, which can erode self-esteem and social comfort.
Beyond its immediate clinical implications, the development of PP405 signals a broader evolution in the science of regenerative medicine. Hair restoration, once dismissed as a superficial concern, is now recognized as a legitimate field of medical inquiry, intersecting with stem cell research, metabolic studies, and pharmaceutical innovation. The molecule’s success could pave the way for similar advancements in other areas of regenerative therapy, from skin rejuvenation to organ repair.
Moreover, the societal implications of accessible hair restoration therapies warrant consideration. As treatments like PP405 become more widely available, they could democratize the pursuit of aesthetic enhancements, leveling the playing field for individuals across socioeconomic boundaries. At the same time, the normalization of such interventions raises questions about beauty standards and the pressures to conform to ideals of youth and vitality.
In the end, UCLA’s discovery of PP405 is more than a scientific milestone—it is a testament to the power of curiosity and innovation to address deeply human concerns. While the molecule’s journey from laboratory to widespread application is still unfolding, its potential to reshape the narrative of hair loss is undeniable. For those who have long sought solutions to the challenges of pattern baldness, PP405 offers not just hope, but the possibility of reclaiming something profoundly personal—the confidence to feel comfortable in one’s own skin.